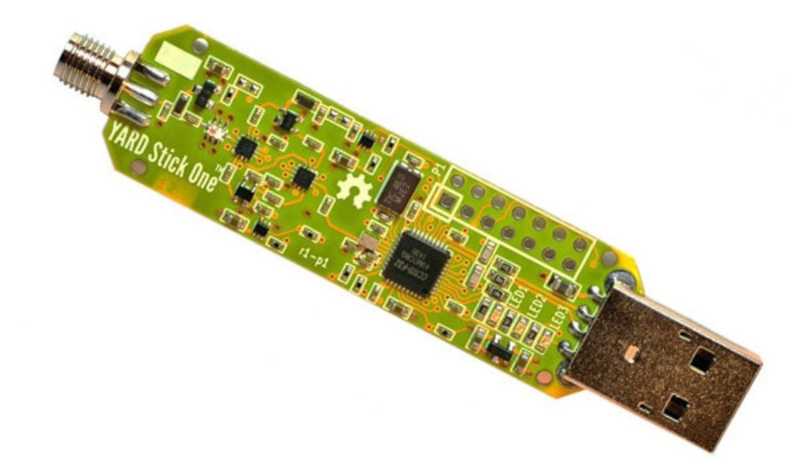
When it comes to SDR, you can usually find cheap products that receive and expensive products that can also transmit. The YARD Stick One bucks that trend. It can send and receive from 300 MHz to 928 MHz, thanks to the onboard TI CC1111 chip. [Wim Ton] on Elektor put the device through its paces. While the frequency range isn’t as broad as some devices, the price is right at about $99. YARD, by the way, stands for Yet Another RF Dongle.
The frequency range isn’t as cut and dry as it might seem. According to the product’s home page: “official operating frequencies: 300 MHz – 348 MHz, 391 MHz – 464 MHz, and 782 MHz – 928 MHz; unofficial operating frequencies: 281 MHz – 361 MHz, 378 MHz – 481 MHz, and 749 MHz – 962 MHz.” The unofficial operating frequencies are not supported by the chip but appear to work in practice.
The device is made for data applications, and the support software is a Python-based interface that abstracts most of what you want to do. You can directly access the device registers if you need more control.
The YARD stick isn’t great as a generic receiver, but as the review points out, you can use it as a transmitter and then grab a cheaper dongle to use as a receiver if you need more capability. The total system cost will still be less than other solutions.
Ultimately, though, [Wim] was less than impressed. Issues with the software and limited documentation didn’t help. But the fact that the CC1111 isn’t meant for general-purpose radio use makes it difficult to put into many projects where you could use an SDR transmitter. A lot of processing happens on the chip which is fine if you know what you want to send and receive ahead of time and the chip supports what you want to do. But for randomly probing and receiving RF, you don’t always have either of those luxuries.
We like the Pluto SDR, which is fairly inexpensive and can transmit. Lime SDR seems to be another popular choice.